the Creative Commons Attribution 4.0 License.
the Creative Commons Attribution 4.0 License.
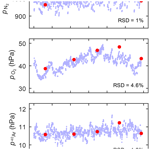
Gas equilibrium membrane inlet mass spectrometry (GE-MIMS) for water at high pressure
Matthias S. Brennwald
Antonio P. Rinaldi
Jocelyn Gisiger
Alba Zappone
Rolf Kipfer
Gas species are widely used as natural or artificial tracers to study fluid dynamics in environmental and geological systems. The recently developed gas equilibrium membrane inlet mass spectrometry (GE-MIMS) method is most useful for accurate and autonomous on-site quantification of dissolved gases in aquatic systems. GE-MIMS works by pumping water through a gas equilibrator module containing a gas headspace, which is separated from the water by a gas-permeable membrane. The partial pressures of the gas species in the headspace equilibrate with the gas concentrations in the water according to Henry's Law and are quantified with a mass spectrometer optimized for low gas consumption (miniRUEDI or similar). However, the fragile membrane structures of the commonly used equilibrator modules break down at water pressures ≳3 bar. These modules are therefore not suitable for use in deep geological systems or other environments with high water pressures. To this end, the SysMoG® MD membrane module (Solexperts AG, Switzerland; “SOMM”) was developed to withstand water pressures of up to 100 bar. Compared to the conventionally used GE-MIMS equilibrator modules, the mechanically robust construction of the SOMM module entails slow and potentially incomplete gas–water equilibration. We tested the gas equilibration efficiency of the SOMM and developed an adapted protocol that allows correct operation of the SOMM for GE-MIMS analysis at high water pressures. This adapted SOMM GE-MIMS technique exhibits a very low gas consumption from the SOMM to maintain the gas–water equilibrium according to Henry's Law and provides the same analytical accuracy and precision as the conventional GE-MIMS technique. The analytical potential of the adapted SOMM GE-MIMS technique was demonstrated in a high-pressure fluid migration experiment in an underground rock laboratory. The new technique overcomes the pressure limitations of conventional gas equilibrators and thereby opens new opportunities for efficient and autonomous on-site quantification of dissolved gases in high-pressure environments, such as in research and monitoring of underground storage of CO2 and waste deposits or in the exploration of natural resources.
- Article
(2633 KB) - Full-text XML
- BibTeX
- EndNote
Gas species are widely used as natural or artificial tracers to study fluid dynamics in environmental and geological systems. The recent development of mobile mass spectrometers for on-site gas analysis has paved the way for efficient on-site gas analysis and monitoring of fluids in environmental systems (Brennwald et al., 2016, 2020; Cassar et al., 2009; Chatton et al., 2017; Gentz and Schlüter, 2012; Kotiaho, 1996; Mächler et al., 2012; Manning et al., 2016; Schlüter and Gentz, 2008; Schmidt et al., 2015; Sommer et al., 2015; Visser et al., 2013). In particular, the gas equilibrium membrane inlet mass spectrometry (GE-MIMS) method used with the miniRUEDI mass spectrometer system (Brennwald et al., 2016; Mächler et al., 2012) provides simple and practical means for accurate quantification of dissolved gas species (e.g., He, Ne, Ar, Kr, Xe, H2, N2, O2, CO2, CH4, C3H8) in lakes, oceans, and groundwaters.
The GE-MIMS method works by pumping water through a gas equilibrator module containing a gas headspace, which is separated from the water by a gas-permeable membrane. The partial pressures of the gas species in the headspace equilibrate with the gas concentrations in the water according to Henry's Law. The partial pressures of the gases are quantified by “sniffing” the gas headspace with the miniRUEDI mass spectrometer without disturbing the gas–water equilibrium. Unlike other membrane inlet methods that rely on the dynamic gas flux across the membrane, the GE-MIMS method operates at gas–water equilibrium and is therefore insensitive to the membrane-specific gas transfer dynamics. Hence, the GE-MIMS method allows accurate quantification of dissolved gas concentrations without the cumbersome and notoriously difficult calibration of the dynamic gas transfer characteristics of the membrane (Brennwald et al., 2016; Mächler et al., 2012).
The combination of the GE-MIMS method with the portable and robust miniRUEDI mass spectrometer allows autonomous on-site quantification of individual gas species during fieldwork at remote locations. The miniRUEDI is therefore widely used with GE-MIMS in environmental and geological research to study gas–water exchange processes, biogeochemical turnover, and the origin and transport of water and other fluids (Batlle-Aguilar et al., 2017; Berndt et al., 2016; Brennwald et al., 2022; Giroud et al., 2023; Knapp et al., 2019; Lightfoot et al., 2022a, b; Mächler et al., 2013a, b; Moeck et al., 2017, 2021; Popp et al., 2019, 2020; Roques et al., 2020; Schilling et al., 2021; Tomonaga et al., 2019; Tyroller et al., 2018; Weber et al., 2018, 2023).
However, the GE-MIMS method is currently limited to applications with water pressures of approximately 3 bar or lower. This limitation is due to the mechanical design of the membrane modules (Liqui-Cel MM 1.7 × 5.5; “LIMM”) that have been tested and validated for use with GE-MIMS. These membrane modules are optimized for efficient gas transfer across the membrane, allowing rapid gas–water equilibration of the gas headspace with the water flowing through the membrane module. The fragile membrane structures used in these modules break down and are irreversibly damaged at high water pressures. Custom-designed pressure-reducing systems have been tested to lower the pressure of the water without affecting its gas concentrations (Weber et al., 2021, 2023; Zappone et al., 2021). However, such systems are expensive, and their operation is challenging and involves a large and poorly constrained dilution of the sample water. The dissolved-gas measurements determined using such pressure-reducing systems therefore tend to exhibit undesired data gaps and large analytical uncertainties. The application of the GE-MIMS method has therefore remained challenging in systems with high water pressures, such as deep geological systems explored for natural resources or for underground storage of waste or CO2.
The recently developed SysMoG® MD membrane module (patent application EP-2210240.2, Solexperts AG, Switzerland; “SOMM”) was designed to withstand water pressures of up to 100 bar. This module might, therefore, allow efficient application of the GE-MIMS method in systems with high water pressures. Owing to its mechanically robust membrane construction, the SOMM module is expected to show much slower and potentially incomplete gas–water equilibration, which would fundamentally affect the correct functioning of the GE-MIMS method (similar as with small dead-end membrane probes as tested by Marion (2022) and Engelhardt (2023)).
Here, we present systematic tests of the gas equilibration efficiency of the SOMM and its potential utility for GE-MIMS. Based on these tests, we developed and validated an adapted GE-MIMS protocol for the accurate quantification of aqueous gas concentrations using the SOMM. This adapted SOMM GE-MIMS technique was implemented in a fluid migration experiment at the Mont Terri Underground Rock Laboratory (Switzerland) to demonstrate its analytical potential for dissolved-gas analysis in high-pressure systems.
2.1 Gas equilibrator modules
The Liqui-Cel LIMM membrane module commonly used for GE-MIMS applications is constructed from a plastic shell (4.3 cm external diameter, 13 cm long) and contains an array of approximately 5000 parallel hollow fibers made of gas-permeable microporous polypropylene (0.3 mm external diameter, 120 mm effective length). The water flow passes through the lumen side of these hollow-fiber membrane elements, and dissolved gases are exchanged with the shell-side gas volume. Depending on the gas species considered, gas–water equilibrium is attained within 5–20 min (Mächler, 2012). The fragile membrane elements and the plastic shell can withstand water pressures of approximately 3 bar but will break down at higher water pressures.
The Solexperts SOMM membrane module uses a stainless-steel shell (6 cm external diameter, 20 cm long) and contains six membrane elements, which are constructed using polymer membrane tubes (12.7 mm external diameter, 86 mm effective length). The water flow passes through the steel shell, and dissolved gases are exchanged with the gas phase in the lumen of the membrane elements. The membrane tubes are internally supported by porous stainless-steel frits to prevent the tubes from collapsing at high water pressures. The gas volumes of the membrane elements are connected in series by stainless-steel tubing to allow purging of the gas through the membrane elements and an external loop for gas sampling.
The dimensions relevant to the GE-MIMS operation with the LIMM and the SOMM are compared in Table 1. Due to the mechanically robust construction of the SOMM, its membrane thickness (W) and the gas-volume-to-membrane-area ratio () are of a magnitude higher than those of the LIMM. Additionally, the non-porous SOMM membrane material is expected to be less permeable to gases than the microporous membrane material of the LIMM (Luis, 2018). As a result, the gas–water equilibration in the SOMM will be substantially slower than in the LIMM and may even be incomplete if gases are consumed rapidly from the headspace for analysis with the miniRUEDI.
2.2 Test setup
Figure 1 shows the test setup used to assess the gas–water exchange dynamics in the SOMM. The SOMM was compared to the LIMM, which serves as a reference for accurate quantification of dissolved gases using the GE-MIMS method (Brennwald et al., 2016; Popp et al., 2019). Both membrane modules were fed with the same water at a flow rate of 1 L min−1. The water was taken from a continuously pumped well in a shallow riparian aquifer near our laboratory (see Popp et al., 2020, for further details). The gas headspaces of the membrane modules were connected to separate gas inlets of a miniRUEDI instrument. The He, Ar, and N2 concentrations in the groundwater are known to be constant, whereas the O2 concentration may change slightly over time (see also Sect. 3.2).
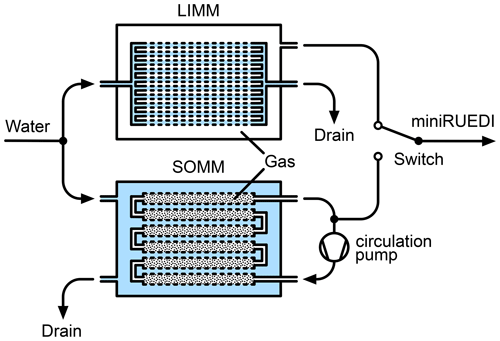
Figure 1Experimental setup to test and compare the LIMM and SOMM membrane modules. Note the reversed gas–water arrangement on the lumen and shell sides of the membrane elements in the LIMM and SOMM.
The gas in the lumen of the SOMM membrane elements and the dead volumes of the connecting tubes was actively purged through an external circulation loop (approx. 2 cm3 internal volume) using a small membrane pump (type FF 20 KTDC-M, KNF Switzerland). In contrast, the LIMM setup does not require purging of the shell side gas, which is connected to the miniRUEDI gas inlet without any dead volumes.
The analytical procedures and configuration of the miniRUEDI instrument followed the recommendations described in Brennwald et al. (2016). The mass-spectrometric peaks of He at , N2 at , O2 at , and Ar at were recorded with the Faraday cup detector. The partial pressures of these gas species in the headspaces of the membrane modules were quantified by peak height comparison relative to a mixture of 95 % atmospheric air and 5 % helium used as a calibration standard.
2.3 Experiment I: gas exchange timescale and gas depletion due to miniRUEDI gas consumption
In a first experiment, we determined the timescale for gas–water exchange in the SOMM and the degree of gas depletion due to gas consumption by the miniRUEDI. To this end, we filled the gas volume of the SOMM with pure He gas at 1 bar. The He was transferred across the membrane and removed by the water flow. At the same time, gas species dissolved in the water were transferred into the SOMM gas volume. The dynamic evolution of the gas species in the in the gas volume was monitored with the miniRUEDI until steady-state conditions were attained. The miniRUEDI was set to continuous sampling, whereby the inlet connection was switched between the two membrane modules at 6.5 min intervals. The relative time fraction (“duty cycle”) spent for gas sampling from a given module was therefore 50 % for both the LIMM and the SOMM.
2.4 Experiment II: modified protocol for unbiased GE-MIMS analysis
In a second experiment, we adapted the GE-MIMS protocol according to the SOMM gas exchange dynamics determined in Experiment I. We decreased the duty cycle of the miniRUEDI gas sampling from the SOMM module with the aim of attaining solubility equilibrium between the water and the gas volume in the SOMM membrane elements. We tested and evaluated the accuracy and precision of this adapted SOMM GE-MIMS technique by comparison with the standard GE-MIMS technique using a LIMM for reference.
3.1 Experiment I
Figure 2 shows the evolution of the He, N2, O2, and Ar partial pressures observed in the SOMM gas volume during Experiment I. The He spike is slowly removed from the SOMM gas volume, whereby the He partial pressure followed an exponential curve. At the same time, N2, O2, and Ar accumulated in the SOMM gas volume. The partial pressures of all gases evolved following first-order kinetics, whereby slightly different time scales to attain steady state were observed for the different gas species. These differences are attributed to differences in (i) the species-specific diffusion kinetics in the membrane material and (ii) the species-specific Henry coefficients controlling the partial-pressure gradients driving the diffusive fluxes of the gas species across the membrane (Luis, 2018). Nevertheless, we conclude that the partial pressures of all gas species attained steady state after approximately 1 d.
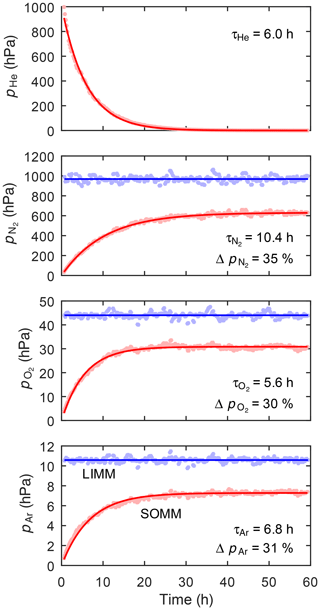
Figure 2Experiment I. Evolution of the partial pressures of He, N2, O2, and Ar in the SOMM module compared to those in the LIMM module. The curved lines correspond to a function of the form or for , where t is time and and τi are the gas-specific curve-fit parameters. The Δpi indicate the depletion of the dynamic steady-state partial pressures in the SOMM () relative to the equilibrium partial pressures in the LIMM.
The steady-state partial pressures of N2, O2, and Ar observed in the SOMM gas volume are 30 %–35 % lower than those in the LIMM, where the partial pressures are at solubility equilibrium with the corresponding dissolved-gas concentrations in the water. The gas depletion in the SOMM module is due to the slow gas–water exchange, which is insufficient to maintain a solubility equilibrium while gas is consumed by the miniRUEDI at a 50 % duty cycle. The gas–water partitioning in the SOMM therefore operates at a dynamic equilibrium between the gas transfer across the membrane and the gas consumption by the miniRUEDI. Also, note the slight fractionation of the different gas species during the dynamic steady state, which is a result of the species-specific rates of gas transfer across the SOMM membrane. Overall, we conclude that with a gas sampling duty cycle of 50 % the SOMM does not attain Henry's Law equilibrium, as it would be required for the GE-MIMS method.
3.2 Experiment II
The characteristic time for the gas–water exchange to attain steady state in the SOMM is approximately T0≈1 d (see Experiment I). The time resolution for repeated dissolved-gas analysis with the SOMM therefore does not need to be higher than one measurement per day. Hence, with a miniRUEDI gas sampling time of Ts≈6.5 min, the duty cycle for gas sampling from the SOMM can be as low as . This much lower duty cycle would result in approximately 100× less gas consumption from the SOMM than with the 50 % duty cycle of Experiment I. The gas depletion in the SOMM gas volume (30 %–35 % in Experiment I) would be reduced by a similar factor and would therefore be expected to be less than the analytical precision of the miniRUEDI (1 %–5 % relative standard deviation, RSD, is typical).
To test the SOMM with the GE-MIMS protocol at a duty cycle of 0.5 %, we increased the intervals for gas sampling from the SOMM to 24 h, keeping the sampling time at 6.5 min. During the remaining 99.5 % of the time, the miniRUEDI sampled the gas from the LIMM headspace. Fig. 3 shows the time series of the partial pressures observed in the gas volumes of the SOMM and LIMM modules. The relative differences between the partial pressures observed in the two equilibrator modules range from 1 %–4.6 %, which is within the analytical uncertainty of the miniRUEDI analysis (Brennwald et al., 2016). The LIMM analysis shows a slight variation in the O2 partial pressures in the groundwater throughout the test period, which is also reflected in the SOMM results. Overall, the results from the SOMM show no bias relative to the LIMM reference. The modified GE-MIMS protocol for the SOMM therefore yields accurate quantification of the dissolved gases in the water, and its analytical uncertainty is in line with that of the standard GE-MIMS technique with the LIMM.
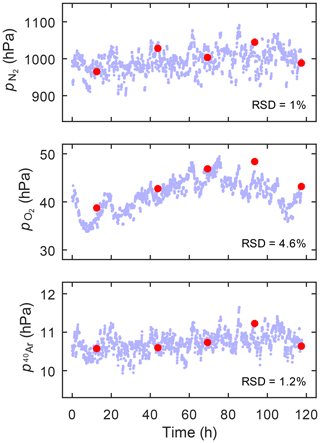
Figure 3Experiment II. Comparison of the N2, O2, and Ar partial pressures determined with GE-MIMS using the LIMM as a reference (small blue dots) and the SOMM with a reduced sampling duty cycle of 0.5 % (large red dots). The RSD values indicate the relative standard deviations of the SOMM data relative to the LIMM data interpolated to the times of the SOMM data points.
3.3 Field demonstration
To demonstrate the performance of a SOMM module with GE-MIMS analysis, we carried out a test in a geological experiment at a borehole in the Mont Terri Underground Rock Laboratory (Switzerland). The Carbon Storage experiment is designed to observe migration of CO2-rich fluids along a fault and their interactions with the surrounding clay rock (Zappone et al., 2021; Weber et al., 2023). After an initial phase of monitoring the natural background conditions, fluids will be artificially injected into the fault at high pressure via an injection borehole. The transport and geochemical evolution of the fluids are analyzed at an observation borehole downstream of the injection point. The water in the observation borehole is circulated at in situ pressure (8.8 bar) in a closed loop to and from the SOMM membrane module. The SOMM water volume and the circulation lines between the module and the observation borehole were filled with water that initially contained dissolved gases at partial pressures close to atmospheric equilibrium. A total of 3 months after starting the water circulation between the observation borehole and the membrane module, a miniRUEDI instrument was connected to the SOMM to test GE-MIMS analysis of the water in the observation borehole as developed in Experiment II. The adapted SOMM GE-MIMS analysis operated fully autonomously during the field test.
Figure 4 shows the time series of the He, N2, O2, and Ar partial pressures determined in the observation-borehole water at daily intervals during a 4-week-long test run. The data gaps in these time series are related to artifacts introduced by manual sampling of the water from the observation borehole. The He partial pressure is approximately 2 orders of magnitude higher than that in air-saturated water (see patm values in Fig. 4), which is due to the accumulation of radiogenic He produced in the host rock. The He partial pressure increased throughout the test period, which indicates that He equilibration between the borehole and the surrounding host rock is still ongoing due to the slow transport of water and solutes within the rock. The N2, O2, and Ar partial pressures do not show any trends throughout the test period and seem to show only small variations (e.g., after the manual water sampling) that are within the analytical uncertainty of the miniRUEDI. The N2 and Ar partial pressures show a slight excess relative to those in air-saturated water, reflecting the typical excess-air signature in groundwater (see Kipfer et al., 2002; Aeschbach-Hertig and Solomon, 2013, for reviews). The low O2 partial pressure in the observation borehole water reflects the anoxic conditions in the porewater surrounding the observation borehole. However, the water in the observation borehole is not fully anoxic, which, similar to He, is attributed to the incomplete equilibration of the initially air-loaded sample water with the anoxic porewater surrounding the observation borehole. Overall, the partial pressures observed with the adapted SOMM GE-MIMS technique correspond to the expected dissolved-gas partial pressures of the water in the observation borehole.
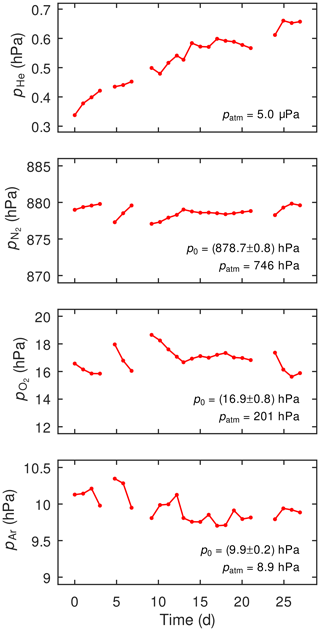
Figure 4Partial pressures of He, N2, O2, and Ar dissolved in the observation borehole water analyzed in the SOMM GE-MIMS field test; p0 indicates the mean and standard deviation of the partial pressure values observed in the time series, while patm indicates the partial pressure of the respective gas in the atmosphere at the elevation of the study site. See text for an explanation of data gaps.
We experimentally determined the dynamics of the gas exchange between the water and the gas volumes in the SOMM membrane module. As expected, the mechanically robust construction of the SOMM membrane elements entails substantially lower gas exchange rates than those achieved with the microporous membrane elements in the LIMM modules commonly used for GE-MIMS. Our tests indicated a characteristic timescale for attaining steady-state gas–water partitioning between the water and the gas volumes in the SOMM module of approximately 1 d, which is about 100× slower than with the LIMM. Also, if the SOMM module is used with the standard protocol for GE-MIMS analysis, we observed a depletion and an elemental fractionation of the gases in the SOMM because the gas transfer across the membrane in the SOMM is insufficient to maintain the gas–water partitioning at solubility equilibrium. The SOMM is therefore not suitable for accurate dissolved-gas quantification using the standard GE-MIMS protocol.
We therefore developed a GE-MIMS protocol that is adapted to the characteristics of the gas exchange dynamics of the SOMM module. Given the slow gas exchange in the SOMM, the time resolution of the GE-MIMS measurements does not need to be higher than one measurement per day. This is in contrast to the standard GE-MIMS protocol with the LIMM, which allows approximately 100 measurements per day using a 100 % gas sampling duty cycle. With the SOMM, GE-MIMS analysis does not require continuous gas sampling to the gas analyzer, which allows using a very low duty cycle for gas sampling to substantially reduce the gas consumption from the SOMM module. The low duty cycle is necessary to avoid the gas depletion in the SOMM and thereby allows the gas–water partitioning in the SOMM module to attain an equilibrium that is controlled only by the specific solubilities of the involved gas species, as required for the GE-MIMS technique. Dissolved-gas measurements from this adapted SOMM GE-MIMS technique showed no bias or extended scatter relative to reference measurements from the standard GE-MIMS protocol using a LIMM. The adapted SOMM GE-MIMS technique therefore allows accurate and precise quantification of dissolved gases. The analytical time resolution that can be achieved with this adapted technique is limited by the SOMM gas–water equilibration time of approximately 1 d. For time series measurements at shorter time intervals, the gas equilibrator module would need to be modified to allow quicker gas–water equilibration and possibly also to provide higher gas–water mass transfer across the membrane to balance the increased gas consumption by the gas analyzer.
We demonstrated the analytical potential of the adapted SOMM GE-MIMS technique in a field test at a deep geological borehole containing water at an in situ pressure of 8.8 bar. The adapted SOMM GE-MIMS technique allowed simple and autonomous quantification of dissolved gases, and therefore overcomes the limitations of previous attempts to apply the GE-MIMS technique in high-pressure water using complex depressurization techniques. The SOMM therefore opens new opportunities for efficient and reliable on-site quantification of dissolved gases in water at high pressures, such as in deep geological systems. While our field demonstration was related to an experiment targeting the migration and fate of injected CO2 and other dissolved gases in the context of CO2 sequestration, the adapted SOMM GE-MIMS technique will also be most suitable for applications in research and monitoring of underground storage of other waste deposits, as well as in the exploration of water, fossil fuels, heat, hydrogen, helium, and other natural resources.
The relevant data are all included in the text, figures, and tables of the manuscript.
Conceptualization: MSB, APR, AZ, RK. Methodology, Data analysis: MSB, APR, RK. Investigation, project administration: MSB, APR. Resources: MSB, APR, JG, AZ. Writing, visualization: MSB. Review and editing: MSB, APR, JG, AZ, RK.
The contact author has declared that none of the authors has any competing interests.
Publisher's note: Copernicus Publications remains neutral with regard to jurisdictional claims made in the text, published maps, institutional affiliations, or any other geographical representation in this paper. While Copernicus Publications makes every effort to include appropriate place names, the final responsibility lies with the authors.
We thank two anonymous reviewers for their helpful comments on the initial submission version of this paper.
This paper was edited by Ralf Srama and reviewed by two anonymous referees.
Aeschbach-Hertig, W. and Solomon, D. K.: Noble Gas Thermometry in Groundwater Hydrology, Springer Berlin Heidelberg, Berlin, Heidelberg, 81–122, https://doi.org/10.1007/978-3-642-28836-4_5, 2013. a
Batlle-Aguilar, J., Banks, E. W., Batelaan, O., Kipfer, R., Brennwald, M. S., and Cook, P. G.: Groundwater residence time and aquifer recharge in multilayered, semi-confined and faulted aquifer systems using environmental tracers, J. Hydrol., 546, 150–165, https://doi.org/10.1016/j.jhydrol.2016.12.036, 2017. a
Berndt, C., Hensen, C., Mortera-Gutierrez, C., Sarkar, S., Geilert, S., Schmidt, M., Liebetrau, V., Kipfer, R., Scholz, R., Doll, M., Muff, S., Karstens, J., Planke, S., Petersen, S., Böttner, C., Chi, W.-C., Moser, M., Behrendt, R., Fiskal, A., Lever, M. A., Su, C.-C., Deng, L., Brennwald, M. S., and Lizarralde, D.: Rifting under steam – How rift magmatism triggers methane venting from sedimentary basins, Geology, 44, 767–770, https://doi.org/10.1130/G38049.1, 2016. a
Brennwald, M. S., Schmidt, M., Oser, J., and Kipfer, R.: A portable and autonomous mass spectrometric system for on-site environmental gas analysis, Environ. Sci. Technol., 50, 13455–13463, https://doi.org/10.1021/acs.est.6b03669, 2016. a, b, c, d, e, f
Brennwald, M. S., Tomonaga, Y., and Kipfer, R.: Deconvolution and compensation of mass spectrometric overlap interferences with the miniRUEDI portable mass spectrometer, MethodsX, 7, 101038, https://doi.org/10.1016/j.mex.2020.101038, 2020. a
Brennwald, M. S., Peel, M., Blanc, T., Tomonaga, Y., Kipfer, R., Brunner, P., and Hunkeler, D.: New Experimental Tools to Use Noble Gases as Artificial Tracers for Groundwater Flow, Frontiers Water, 4, 925294, https://doi.org/10.3389/frwa.2022.925294, 2022. a
Cassar, N., Barnett, B. A., Bender, M. L., Kaiser, J., Hamme, R. C., and Tilbrook, B.: Continuous High-Frequency Dissolved O2/Ar Measurements by Equilibrator Inlet Mass Spectrometry, Anal. Chem., 81, 1855–1864, https://doi.org/10.1021/ac802300u, 2009. a
Chatton, E., Labasque, T., de La Bernardie, J., Guihéneuf, N., Bour, O., and Aquilina, L.: Field Continuous Measurement of Dissolved Gases with a CF-MIMS: Applications to the Physics and Biogeochemistry of Groundwater Flow, Environ. Sci. Technol., 51, 846–854, https://doi.org/10.1021/acs.est.6b03706, 2017. a
Engelhardt, E.: Stories of Noble Gases in Low Permeable Sediments, Hydrothermal Systems, and Groundwater in the Sea, Doctoral thesis, ETH Zurich, Zurich, https://doi.org/10.3929/ethz-b-000599686, 2023. a
Gentz, T. and Schlüter, M.: Underwater cryotrap-membrane inlet system (CT-MIS) for improved in situ analysis of gases, Limnol. Oceanogr., 10, 317–328, https://doi.org/10.4319/lom.2012.10.317, 2012. a
Giroud, S., Tomonaga, Y., Brennwald, M. S., Takahata, N., Shibata, T., Sano, Y., and Kipfer, R.: New experimental approaches enabling the continuous monitoring of gas species in hydrothermal fluids, Frontiers Water, 4, https://doi.org/10.3389/frwa.2022.1032094, 2023. a
Kipfer, R., Aeschbach-Hertig, W., Peeters, F., and Stute, M.: Noble gases in lakes and ground waters, in: Noble gases in geochemistry and cosmochemistry, edited by: Porcelli, D., Ballentine, C., and Wieler, R., vol. 47 of Rev. Mineral. Geochem., Mineralogical Society of America, Geochemical Society, 615–700, https://doi.org/10.2138/rmg.2002.47.14, 2002. a
Knapp, J., Osenbrück, K., Brennwald, M. S., and Cirpka, O. A.: In-situ mass spectrometry improves the estimation of stream reaeration from gas-tracer tests, Sci. Total Environ., 655, 1062–1070, https://doi.org/10.1016/j.scitotenv.2018.11.300, 2019. a
Kotiaho, T.: On-site environmental and in situ process analysis by mass spectrometry, J. Mass Spectrom., 31, 1–15, https://doi.org/10.1002/(SICI)1096-9888(199601)31:1<1::AID-JMS295>3.0.CO;2-J, 1996. a
Lightfoot, A. K., Brennwald, M. S., Prommer, H., Stopelli, E., Berg, M., Glodowska, M., Schneider, M., and Kipfer, R.: Noble gas constraints on the fate of arsenic in groundwater, Water Res., 214, 118199, https://doi.org/10.1016/j.watres.2022.118199, 2022a. a
Lightfoot, A. K., Stopelli, E., Berg, M., Brennwald, M., and Kipfer, R.: Noble gases in aquitard provide insight into underlying subsurface stratigraphy and free gas formation, Vadose Zone J., 22, e20232, https://doi.org/10.1002/vzj2.20232, 2022b. a
Luis, P., ed.: Fundamental Modelling of Membrane Systems, Elsevier, https://doi.org/10.1016/C2016-0-02489-0, 2018. a, b
Mächler, L.: Quantification of oxygen turnover in groundwater by continuous on-site gas concentration measurements, PhD thesis, ETH Zürich, https://doi.org/10.3929/ethz-a-009777572, 2012. a
Manning, C. C., Stanley, R. H. R., and Lott, D. E. I.: Continuous Measurements of Dissolved Ne, Ar, Kr, and Xe Ratios with a Field-Deployable Gas Equilibration Mass Spectrometer, Anal. Chem., 88, 3040–3048, https://doi.org/10.1021/acs.analchem.5b03102, 2016. a
Marion, C.: Measuring dissolved gases in tree xylem sap, Master's thesis, ETH Zurich, https://www.research-collection.ethz.ch/handle/20.500.11850/607722 (last access: 11 January 2024), 2022. a
Moeck, C., Radny, D., Popp, A., Brennwald, M. S., Stoll, S., Auckenthaler, A., Berg, M., and Schirmer, M.: Characterization of a managed aquifer recharge system using multiple tracers, Sci. Total Environ., 609, 701–714, https://doi.org/10.1016/j.scitotenv.2017.07.211, 2017. a
Moeck, C., Popp, A. L., Brennwald, M. S., Kipfer, R., and Schirmer, M.: Combined method of 3H 3He apparent age and on-site helium analysis to identify groundwater flow processes and transport of perchloroethylene (PCE) in an urban area, J. Contam. Hydrol., 238, 103773, https://doi.org/10.1016/j.jconhyd.2021.103773, 2021. a
Mächler, L., Brennwald, M. S., and Kipfer, R.: Membrane Inlet Mass Spectrometer for the Quasi-Continuous On-Site Analysis of Dissolved Gases in Groundwater, Environ. Sci. Technol., 46, 8288–8296, https://doi.org/10.1021/es3004409, 2012. a, b, c
Mächler, L., Brennwald, M. S., and Kipfer, R.: Argon Concentration Time-Series As a Tool to Study Gas Dynamics in the Hyporheic Zone, Environ. Sci. Technol., 47, 7060–7066, https://doi.org/10.1021/es305309b, 2013a. a
Mächler, L., Peter, S., Brennwald, M. S., and Kipfer, R.: Excess air formation as a mechanism for delivering oxygen to groundwater, Water Resour. Res., 49, 6847–6856, https://doi.org/10.1002/wrcr.20547, 2013b. a
Popp, A., Scheidegger, A., Moeck, C., Brennwald, M. S., and Kipfer, R.: Integrating Bayesian Groundwater Mixing Modeling With On‐Site Helium Analysis to Identify Unknown Water Sources, Water Resour. Res., 55, 10602–10615, https://doi.org/10.1029/2019WR025677, 2019. a, b
Popp, A., Manning, C., Brennwald, M. S., and Kipfer, R.: A New in Situ Method for Tracing Denitrification in Riparian Groundwater, Environ. Sci. Technol., 54, 1562–1572, https://doi.org/10.1021/acs.est.9b05393, 2020. a, b
Roques, C., Weber, U. W., Brixel, B., Krietsch, H., Dutler, N., Brennwald, M. S., Villiger, L., Doetsch, J., Jalali, M., Gischig, V., Amann, F., Valley, B., Klepikova, M., and Kipfer, R.: In situ observation of helium and argon release during fluid-pressure-triggered rock deformation, Nat. Sci. Rep., 10, p7347, https://doi.org/10.1038/s41598-020-63458-x, 2020. a
Schilling, O. S., Parajuli, A., Tremblay Otis, C., Müller, T. U., Antolinez Quijano, W., Tremblay, Y., Brennwald, M. S., Nadeau, D. F., Jutras, S., Kipfer, R., and Therrien, R.: Quantifying Groundwater Recharge Dynamics and Unsaturated Zone Processes in Snow-Dominated Catchments via On-Site Dissolved Gas Analysis, Water Resour. Res., 57, e2020WR028479, https://doi.org/doi.org/10.1029/2020WR028479, 2021. a
Schlüter, M. and Gentz, T.: Application of membrane inlet mass spectrometry for online and in situ analysis of methane in aquatic environments, J. Am. Soc. Mass Spectr., 19, 1395–1402, https://doi.org/10.1016/j.jasms.2008.07.021, 2008. a
Schmidt, M., Linke, P., Sommer, S., Esser, D., and Cherednichenko, S.: Natural CO2 Seeps Offshore Panarea: A Test Site for Subsea CO2 Leak Detection Technology, Mar. Technol. Soc. J., 49, 19–30, https://doi.org/10.4031/MTSJ.49.1.3, 2015. a
Sommer, S., Schmidt, M., and Linke, P.: Continuous inline mapping of a dissolved methane plume at a blowout site in the Central North Sea UK using a membrane inlet mass spectrometer – Water column stratification impedes immediate methane release into the atmosphere, Mar. Petrol. Geol., 68, 766–775, https://doi.org/10.1016/j.marpetgeo.2015.08.020, 2015. a
Tomonaga, Y., Grioud, N., Brennwald, M. S., Horstmann, E., Diomidis, N., Kipfer, R., and Wersin, P.: On-line monitoring of the gas composition in the Full-scale Emplacement experiment at Mont Terri (Switzerland), Appl. Geochem., 100, 234–243, https://doi.org/10.1016/j.apgeochem.2018.11.015, 2019. a
Tyroller, L., Brennwald, M. S., Busemann, H., Maden, C., Baur, H., and Kipfer, R.: Negligible fractionation of Kr and Xe isotopes by molecular diffusion in water, Earth Planet. Sc. Lett., 492, 73–78, https://doi.org/10.1016/j.epsl.2018.03.047, 2018. a
Visser, A., Singleton, M. J., Hillegonds, D. J., Velsko, C. A., Moran, J. E., and Esser, B. K.: A membrane inlet mass spectrometry system for noble gases at natural abundances in gas and water samples, Rapid Commun. Mass Sp., 27, 2472–2482, https://doi.org/10.1002/rcm.6704, 2013. a
Weber, U., Cook, P., Brennwald, M. S., Kipfer, R., and Stieglitz, T.: A Novel Approach To Quantify Air–Water Gas Exchange in Shallow Surface Waters Using High-Resolution Time Series of Dissolved Atmospheric Gases, Environ. Sci. Technol., 53, 1463–1470, https://doi.org/10.1021/acs.est.8b05318, 2018. a
Weber, U., Rinaldi, A., Roques, C., Zappone, A., Bernasconi, S., Jaggi, M., Wenning, Q., Schefer, S., Brennwald, M. S., and Kipfer, R.: Geochemical Monitoring of a CO2 Injection into a Caprock Analogue, in: Goldschmidt Conference 2021, virtual conference, p. 7347, https://doi.org/10.7185/gold2021.7347, 2021. a
Weber, U. W., Rinaldi, A. P., Roques, C., Wenning, Q. C., Bernasconi, S. M., Brennwald, M. S., Jaggi, M., Nussbaum, C., Schefer, S., Mazzotti, M., Wiemer, S., Giardini, D., Zappone, A., and Kipfer, R.: In-situ experiment reveals CO2 enriched fluid migration in faulted caprock, Sci. Rep., 13, 17006, https://doi.org/10.1038/s41598-023-43231-6, 2023. a, b, c
Zappone, A., P., R. A., Grab, M., Wenning, Q. C., Roques, C., Madonna, C., Obermann, A. C., Bernasconi, S. M., Brennwald, M. S., Kipfer, R., Soom, F., Cook, P., Guglielmi, Y., Nussbaum, C., Giardini, D., Mazzotti, M., and Wiemer, S.: Fault sealing and caprock integrity for CO2 storage: an in situ injection experiment, Solid Earth, 12, 319–343, https://doi.org/10.5194/se-12-319-2021, 2021. a, b